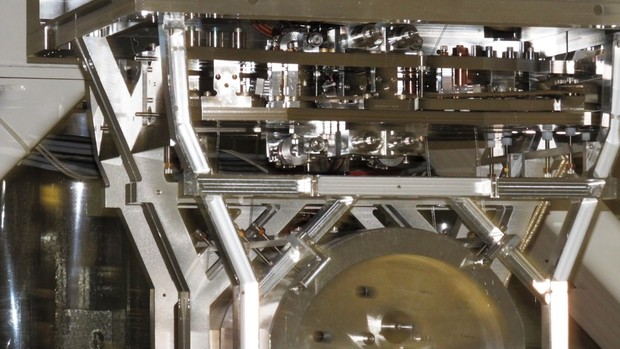
The upper section of one of LIGO's complex suspension systems. (Credit: Caltech/MIT/LIGO Lab)
LIGO Technology
Below you can learn a little more about some of the U.S. National Science Foundation Laser Interferometer Gravitational-wave Observatory's (NSF LIGO) primary engineering technology systems.
For a deeper dive into how all these systems work, visit Look Deeper
Seismic Isolation – Suspending the Mirrors |
||
Since detecting gravitational waves ultimately depends on the absolute stillness of NSF LIGO’s test-masses (which reflect LIGO’s main laser and tell us if a gravitational wave has passed), these mirrors reside at the end of a series of complex mechanical vibration isolation systems designed to make them ‘feel’ like they are floating in space. Passive Vibration Reduction The largest of LIGO's mirrors, the 'test masses' hang at the bottom of massive 4-segment pendulum structures known as "quad" suspensions situated at the beginning and end of each of LIGO's arms (figure at right). Quads rely heavily on passive methods of reducing vibrations. While some sensors and actuators help to keep the suspension steady, much of the vibration isolation is achieved with two basic principles: the fundamental properties of pendulums and the law of inertia. First, by their very nature, each link in a pendulum absorbs some vibrations arriving from above and prevents them from being passed to the link below. Furthermore, by placing heavy masses at each link, the law of inertia comes into play: the heavier the mass, the less it wants to move and the less vibration it will pass to the mass below. The top two masses are metal, each weighing 20kg (44lb), while the third and fourth are pristine silica glass cylinders each weighing 40kg (88 lbs): the fourth mass is the test mass. Combined, this structure is so effective at reducing vibrations that any present at the top of the suspension are made 100-million-times smaller by the time they reach the test mass itself! While quads are the largest suspension systems LIGO employs, smaller suspension systems are used throughout the interferometer, each helping to eliminate terrestrial vibrations. Active Vibration Reduction As astoundingly effective as the quad is, on its own, it cannot eliminate enough vibration to allow LIGO to sense gravitational waves. For this to happen, the quad is attached to a larger structure whose job is to remove vibrations caused by things like earthquakes, traffic, or even the tides, before they have a chance to affect the pendulum. This system is known as the "active isolation" system (photo at right). It functions much like noise canceling headphones: Seismometers around each observatory sense a range of ground motions, then send these signals to a computer that combines them and determines counter motions. The superstructure is then physically moved with hydraulics in a way that cancels-out external vibrations before they touch the pendulum. Any vibrations not removed by the active system are finally removed by the quad suspension. Working together, these passive and active damping systems ensure that LIGO's test masses are isolated from as much terrestrial vibration as is physically possible, leaving them still enough to sense the fleeting changes in spacetime caused by a passing gravitational wave. |
||
|
Optics |
||
Since gravitational waves are so inconceivably small, and photons themselves tell us when a gravitational wave has passed, every photon that makes its way to the photodetector is valuable. This means that LIGO’s mirrors and other “optics” need to be extra special. LIGO “optics” include the test masses and the laser beam-splitter (both of which are housed in large suspension systems), and all other small mirrors that LIGO’s laser encounters. LIGO's largest optics are the test masses, the mirrors that reflect the laser beam within the arms of the interferometer. They are called 'test masses' because they respond to the vibrations in spacetime caused by a passing gravitational wave, i.e., they 'test' for vibrations. Weighing 40kg each, and hanging at the bottom of the quad suspensions, test masses are made of ultra-pure materials laid down in nano-meter thick layers. They are coated with coatings that reflect all but one of every 5 million photons that hit them! |
||
The test masses also refocus the laser, preventing it from continually spreading out as it travels. This is important because the laser inside each arm travels over 1000km before merging with the beam from the other arm and heading to the photodetector. If the beam continually spread out, little light would strike the photodetector and the tell-tale flicker of light caused by a gravitational wave would be too faint to sense. The beam splitter is also a crucial component. This mirror splits the initial laser beam in half, sending one half straight into an arm while reflecting the other half 90-degrees to travel down the other arm. The beams then bounce between the test masses in each arm about 300 times before they remerge at the beam splitter and are directed to the photodetector. Without these remarkable pieces of glass preserving and redirecting nearly every photon that strikes them, LIGO could never detect gravitational waves. |
Ultra-High Vacuum |
||
Another way that we enable LIGO to detect gravitational waves is to house almost all of the instrument’s components within an ultra-high vacuum. The air was pumped out of the interferometers' arms in 1998 (LHO) and 1999 (LLO). It took 40 days to achieve a vacuum clean enough for LIGO to operate, and we have maintained this level of vacuum ever since. The atmospheric pressure inside LIGO's arms is one-trillionth that of sea level, which means there are about 10 million molecules per cubic centimeter. While that sounds like a lot, it is actually one of the cleanest and largest ultra-high vacuums in the world. |
||
Why so extreme? The effects of gravitational waves on LIGO’s laser are so unimaginably small that anything interfering with the laser beam could cause the beam to scatter (reducing the number of photons reaching the photodetector) or flicker in a way that mimics or obscures a gravitational wave signal. To avoid such phantom flickers, LIGO’s laser and all of its optical components must operate in as clean a vacuum as possible. Operating in a vacuum also removes the chance of dust landing on one of LIGO’s optical components. If the tiniest bit of dust landed on one of the mirrors and was struck by the laser, it would be incinerated causing irreversible damage to the mirror’s surface. Since each of LIGO’s test mass mirrors costs about $2 Million to produce, avoiding this damage by maintaining one of the cleanest vacuum systems on Earth is critical to our operation.
|
Laser |
||
LIGO detects gravitational waves by generating a laser beam, splitting it in half, and sending each half down an arm of the interferometer. Inside each arm, the laser bounces back and forth between test masses about 300 times before the two beams are recombined. The recombined beam is then sent to a photodetector, which monitors the intensity of the exiting beam. In this way, the laser acts as a ruler. As long as the arms don’t change length, when the two beams are recombined, they cancel each other out and the photodetector senses nothing. But in the presence of a passing gravitational wave, the arms oscillate in length, causing the lasers to travel slightly different distances. When the beams are recombined, their waves are out of sync, and instead of cancelling each other out, they generate a flicker of light at the photodetector. (The animation below shows a simplified version of this process.) Gravitational waves generate predictable oscillations and hence patterns of flickers, which LIGO’s computers hunt for in the data that continually streams from the instrument. |
||
To detect gravitational waves, we must constantly measure the length of each arm to within a single wavelength of light! The only way to do this is with a laser that consistently and reliably emits light of a single, known wavelength. If the wavelengths of light emitted by the laser varied, then measuring the lengths of the arms to the level of precision needed would be like using a meter stick whose millimeter markings randomly changed their spacing; you could never make an accurate measurement! Regular commercial lasers are not this reliable, so LIGO's laser was specially engineered to be one of the most stable, pristine lasers of its kind ever invented. Without LIGO's special ultra-stable and clean laser, detecting gravitational waves would be impossible. |
Simplified illustration of how we use lasers to tell is if a GW has passed |